Not all renewable energy sources are equal in the media spotlight. Although it is the leading renewable energy source in the world, biomass is not the most studied. The following is therefore of great importance for anyone who desires to understand what bio-sourced energy sources, or bio-energy, are and what their possible developments are.
The ecological transition that is now being imposed on all societies, especially industrialized ones, requires an increasing use of renewable energy sources. Among them, alongside new techniques for producing electricity, biomass – historically used since the beginning of humanity – occupies a major place.
But what is biomass? Originating mainly from agriculture, livestock, forests and the sea, it is relatively unknown under this generic name because each of these fields provides very different final resources, from food products to the most varied materials and energy sources used for lighting, cooking, and especially fuels for heating.
To fully understand the place taken and to be taken by biomass products in an energy balance (Read: The energy balance), it is essential to go back to the definition of biomass, its energy properties, its recovery cycle and the various forms of its contribution to meeting energy needs (Read: Energy needs).
1. What is biomass?
Biomass, at the heart of the living world, and therefore the essential substrate of the biosphere, is produced by plants, animals, insects and micro-organisms, mainly during their growth. Its fundamental characteristic is that it consists of organic, vegetable or animal matter, or at least of vegetable or animal origin; examples are fossil sediments that are now inert (hydrocarbons), or that are no longer alive but nevertheless inhabited by active micro-organisms, which characterizes residues, waste and other fermentable materials that, under the action of certain bacteria, are then called biodegradable[1].
The essential chemical characteristic of biomass is to be built from carbonaceous molecules, structured according to innumerable formulas, almost always of the polymer type, belonging to families well known in organic chemistry (polysaccharides, or lipids, for example). In these assemblies of components, mineral elements are generally incorporated in small quantities, which are found in the ashes of burnt products.
1.1. Legal definition
Since the “Grenelle de l’environnement” conference (September-November 2007) and the programming law n° 2009-967, biomass is legally defined in France as “the biodegradable fraction of products, waste and residues from agriculture, including plant and animal substances from land and sea, forestry and related industries, as well as the biodegradable fraction of industrial and household waste” (Figure 1).
This definition was inspired by the one previously adopted by Directive 2001-77-EC of the European Parliament and endorsed by the Council on 27 September 2001, with a view to specifying the nature of renewable energy sources used for electricity production. It is therefore a reference point for European domestic regulation.
For the “biomass” resource to be characterized as a renewable material, it is “assumed that the plant grows back after being harvested”, which implies that its stock is managed in a sustainable way without decreasing over time, thus keeping its incorporated carbon quantity stable.
1.2. Biomass constituent resources
In general, the biomass resources available on our planet, derived from large production areas, can be classified as follows:
- products from agriculture (wheat, maize, potatoes, beetroot, sugar cane, rapeseed, sunflower, soya, palm and others) and livestock (fats in particular), all initially dedicated at least to food or feed, to which are added plants dedicated to energy crops, such as giant miscanthus for bioethanol, switchgrass or rapeseed for biodiesel
- agricultural and livestock co-products and residues: straw, pulp, grains, oilcake, cattle manure, pig manure, poultry droppings, etc.
- fisheries resources: animal products from the sea and wetlands and their waste, algae and microalgae (the latter promising a great future because they are very rich in energy)
- forest wood, which provides most of the wood energy resources used for cooking food, heating homes and communities, as well as energy tree plantations such as poplar, pine, eucalyptus or short-rotation coppice (SRT, i.e. a few years), including willows
- natural waste from wood and forestry (chips, sawdust) as well as from construction wood (chips, sawdust) and packaging wood industries (crates, pallets, cooperage), except those treated with toxic chemicals
- waste from the food industry, housing and urban communities, often wet or even liquid, including sewage sludge, household waste and organic waste from waste collection centers, waste from distribution and coffee shops or from green spaces
2. Biomass contribution to energy supply
Biomass has been used by animals and humans since the beginning of their presence on earth to satisfy three main basic needs: food, materials and energy in various forms (Read: Energy consumption before the industrial era).
2.1. From primary resources to useful resources
Resources are products of nature, but they are rarely directly consumable: food must be peeled, ground, cooked; energy sources captured and processed by converters such as charcoal wheels or water or windmills; materials (wood, wool or leather) processed with tools.
These transformation successions (Figure 2) form chains that will transform from:
- primary resources, naturally accessible, extracted or cultivated (solar radiation, wind, hydropower, geothermal energy, nuclear energy, and chemical energy contained in plants and animals)
- to final products (logs, pellets, sludge, gas, fuels, electricity), themselves transformed into useful energy sources deployed for all biological (metabolism), mechanical (driving force of machines, vehicles), thermal and chemical uses.
These transformations take place with very variable yields depending on the nature of the resource, its moisture content, its energy density and the transformation processes used, but obviously with a consumption yield that is always less than 1 (= 100%).
In general, biomass valorization therefore consists in transforming primary resources in order to obtain final products corresponding to the various material needs of consumers (food, materials and energy), within different sectors. In the case of biomass energy, energy is exchanged in many forms, in its production flow and in interaction with its environment.
2.2. Biomass as a primary energy resource
Both globally and in most countries, especially those with little industrialization, biomass remains by far the leading renewable energy source in the consumption of primary energy sources (Figure 3).
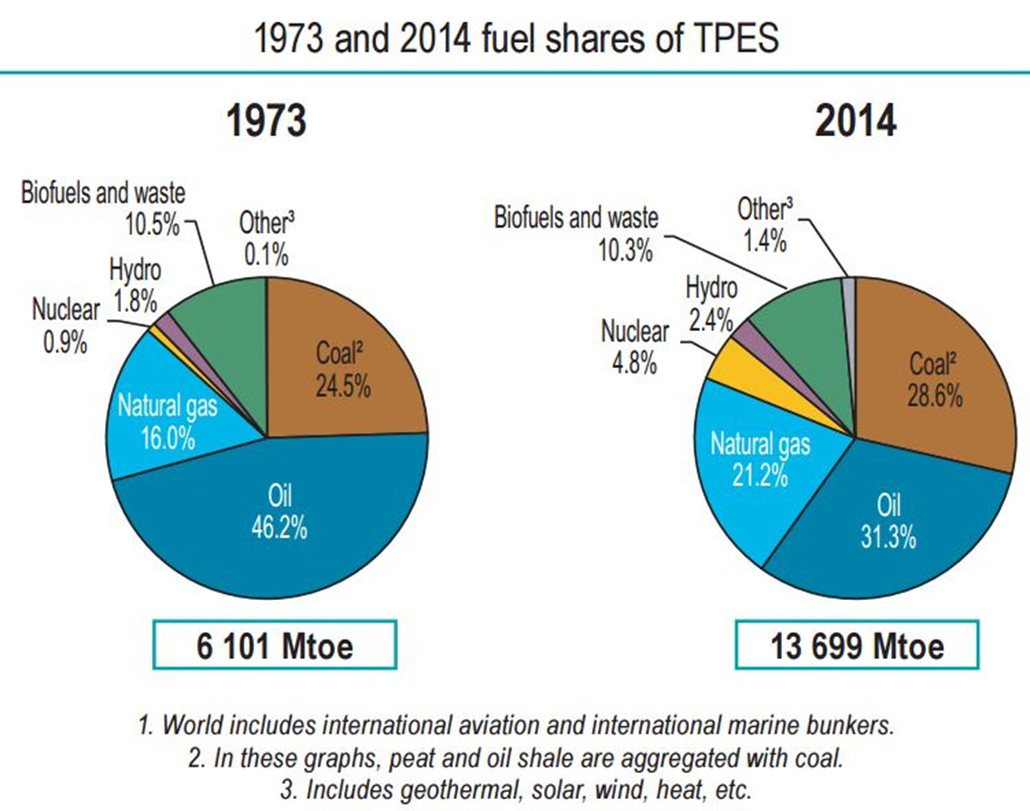
Fig. 3. Evolution of the structure of the world energy balance. Biomass is called biofuel and waste. Remember that Mtoe (millions of tons of oil equivalent) are Mtep (millions of tons of oil equivalent) . [Source: OECD/IEA 2016 Key World Energy Statistics, IEA Publishing]
Excluding food, not considered as an energy product, it represents about 10% of all primary sources, behind oil, coal and natural gas but ahead of nuclear, hydroelectricity and other renewable sources. However, this share can vary considerably from one region of the world to another, due to differences in geographical situations, natural resources and, above all, levels of development (Table 1).
Table 1:%of primary energy consumption in various regions of the world
World | 10,4 | China | 7,1 |
OCDE member states | 5,7 | India | 23,5 |
Japan | 2,5 | Brazil | 27,7 |
USA | 4,7 | Africa | 47,6 |
Europe | 8,2 | Sub-Saharan Africa | 61,0 |
OCDE non-member states | 13,9 | West Africa | 74,6 |
Middle East | 0,1 | Central Africa | 78,4 |
Russia | 1,1 | East Africa | 84,8 |
Source: IEA, World Energy Outlook 2016 and Africa Energy Outlook 2014. |
Compared to renewable sources alone, the share of biomass is almost always predominant, as in the case of France (Figure 4).
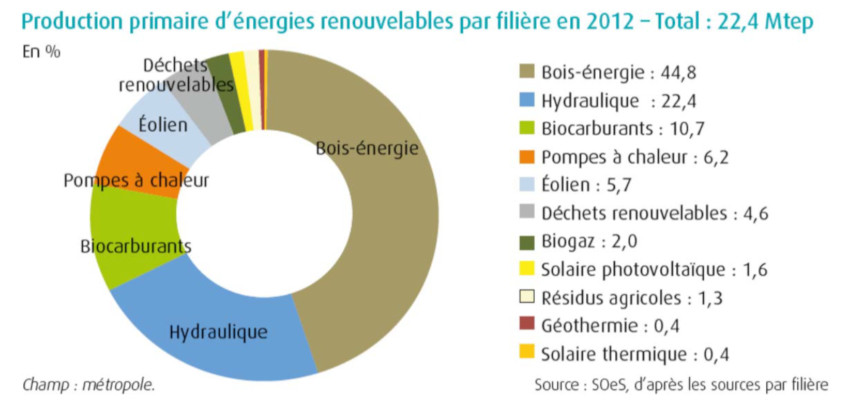
Fig. 4. Share of biomass in the production of renewable sources in France [Source: Senate / National Assembly Report – February 10, 2016, Key figures on renewable energies]
Like all renewable energy sources, 98% of which come from the sun, biomass energy comes from solar radiation.
Almost all renewable energies are carbon-free: heat absorbed by the earth, the oceans and the biosphere, energy that can be used (indirectly) in the form of hydroelectric, wind or marine energy, and energy that can be used directly in solar, thermal or photovoltaic collectors in the form of electricity (Read: Solar energy: the theoretical basis for understanding it).
Another part is carbonated. This is the share of solar energy absorbed by biomass, including marine biomass, and transformed into stored chemical energy. It resides mainly in plants, as well as in living, marine, plant and animal beings, mainly algae and plankton. It is found in abundance in wood, shrubs, coppices or grass (Read: Photosynthesis and biomass).
A traditional source of energy since the beginning of humanity, this biomass energy is currently massively consumed in southern countries (Africa, Asia) to satisfy domestic needs for cooking food and heating homes. In particular, charcoal is still widely used. But advanced countries also use it because of its natural abundance (wood from Canada’s forests), or by planting forests (pines, eucalyptus, oil palms), or by exploiting crops such as sugar cane, an important fuel source in Brazil.
2.3. Biomass resources as energy sources
Biomass resources can all become energy sources. Almost all of them are highly diversified and can be transformed into fuels, including wet organic materials such as slaughtered plant and animal tissues, feces and waste water, but of all these resources, it is the dry matter that counts most.
a/ First, all direct energy uses are found in the form of fuels: firewood (logs) and natural forest residues, coppice and crops such as forest chips, straw, barley grains, grape and corn stalks, beet pulp, sunflower shells and others. However, it is not desirable to burn agricultural residues, which are rich in organic matter, particularly protein.
b/ Fuel products derived from wood, which have therefore undergone artisanal or industrial transformations, are also available: charcoal, pellets, sawdust, including for urban heat production and electro-generation. Their economic value is certain.
c/ There are also plant crops (non-food) for energy production (heat, electricity) or possibly for biogas production. They are called “dedicated energy crops”. These are generally fast-growing plants (willows, pines, eucalyptus, miscanthus, switchgrass, sorghum). These crops are not yet very developed, as their economic interest is not always demonstrated for energy purposes, although it can be demonstrated in the production of materials such as pulp.
d/ Food crops, which are very diverse, are all resources with the potential to become energy commodities. Food is by nature an energy product essential for the survival and physical and intellectual activities of all living beings, humans, animals and plants. Most of the crop products found in the agro-industry can therefore be transformed into energy products, mainly fuels: cereal starch or sugar from beet or sugar cane into bio-ethanol, oilseed oil (sunflower, rape, soybean) into biodiesel, etc.
Corn and other plants, including exotic plants, can also be grown to produce biogas by methanization. This gas can be consumed in industry, distributed in networks or used as fuel in vehicles.
These energy transformations of food products are nowadays very developed in some countries such as the United States (maize), Germany (maize), or Brazil (sugar cane), but disputed insofar as their cultivation economically disrupts markets whose prices can be distorted and risks creating food shortages.
e/ With a vocation neither for energy nor for food, some crops produce utilitarian materials in countless forms: fiber plants such as cotton, flax, hemp, dedicated plants (rubber), medicinal plants, perfume plants, and even a priori food plants (such as potatoes), but used for very diverse polymer productions, particularly for the manufacture of plastics and multiple fine chemical products (cosmetics, solvents, additives, detergents, adhesives, insulation, colors and paints). The products of these crops are generally not used for energy purposes.
f/ Waste of all kinds, on the other hand, are increasingly transformed into energy materials, in particular for the production of biogas, by fermentation (methanization) or methanation.
g/ There are also algae, primarily a food source for many Asian populations and used quite widely in the Western world (spirulina, for example), especially as an ingredient for pharmaceutical and cosmetic uses. Microalgae, which are now the subject of numerous studies and experiments (particularly by aquaculture techniques) are known for their remarkable energy properties, due to their very high content of oilseeds. For this reason, they are considered to be an important potential source of biofuels (biodiesel), but only in the next 15 to 20 years (Figure 5).
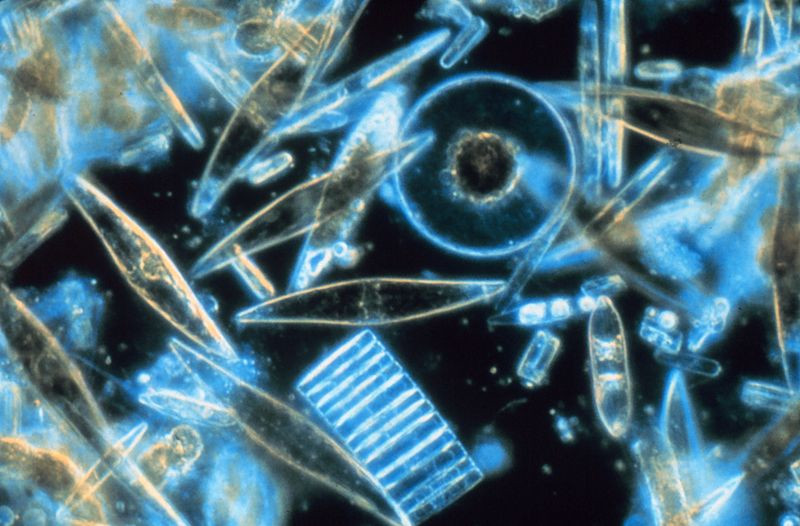
Fig. 5. Marine biomass includes diatoms, brown single-celled algae living in the oceans and forming part of phytoplankton. [Source : Prof. Gordon T. Taylor, Stony Brook University / Public domain]
3. Energy properties of biomass
Where does the energy in biomass come from? It originates from photosynthesis, but it then depends on the energy content of the various biomass resources, which itself produces transformation yields.
3.1. Photosynthesis
Thanks to chlorophyll pigments, plants produce their biomass through photosynthesis, a process in which organic molecules (carbohydrates, especially glucose, sugar monomer) are produced under the action of solar radiation, by absorption of carbon dioxide from the atmosphere and reaction on water molecules, simultaneously releasing oxygen into the atmosphere.
Light energy + 6 CO2 + 6 H2O to C6H12O6 + 6 O2
This solar energy is thus converted and stored in part as chemical energy, constituting a useful reserve for the plant’s vital functions, including its nutrition and respiration. This is done in the absence of light at night, by consuming oxygen and releasing carbon dioxide, in a combustion reaction similar to that of the respiration of animals and humans, which releases heat (Read: Photosynthesis and biomass).
This complete photosynthesis / respiration (combustion) cycle is carbon neutral, as the CO2 released into the atmosphere during combustion has previously been absorbed by the plant in photosynthesis. Unlike fossil fuel combustion, over a period of time that allows plants to regenerate, there is no CO2 emission in the use of wood energy.
Depending on the plant species considered, photosynthesis develops various molecules important in the plant’s energy storage, including sucrose (glucose polymer sugar), starch (an even more complex polymer, found mainly in cereals), glycerol (found in oilseeds and rich in energy) and especially cellulose, a major component of wood and herbaceous plants that is also rich in fiber (cotton). In wood, this molecule is associated with hemi-celluloses and lignin.
Algae and microalgae also absorb and store considerable amounts of carbon through photosynthesis, with the oceans occupying about 70% of the Earth’s surface.
Quantitatively and globally, photosynthetic reactions absorb at least 100 billion tons (Gt) of carbon annually, storing about 100 billion tons of oil equivalent (Gtoe) of energy, 59 of which is for terrestrial vegetation, which corresponds to 8 times the global energy consumption, in the order of 12 Gtoe[2].
The solar energy scattered on the earth (including the oceans) is gigantic: on average about 0.3 kW per m2, which corresponds to 3000 kW per hectare or 300,000 kW per km2. But a very small part of this energy is actually transformed by photosynthesis, less than 1/1,000th of the quantity received, due to the effect of many physical factors (heat loss and light spectrum-related losses in particular), chemical (auxiliary reactions), environmental (temperature, presence of water), dispersion and orientation of plants and leaves, among others.
Although, under the most favorable conditions, the energy recovered by photosynthesis can reach 5 to 6% of the energy captured; it ultimately represents on average significantly less than 1%. By way of comparison, photovoltaic cells offer yields of up to about 20%. Biomass is therefore a very abundant but extremely diffuse source of energy!
3.2. Plant material yield and energy content
To understand the energy efficiency of biomass, it is first necessary to consider the quantities of plant matter produced by plants in space and time, and therefore their material efficiency. This quantity depends strongly on the nature of the plant, but also on the climate, the availability of water and nutrients, as well as the methods of cultivation and even predation.
Gross biomass production (Pb) in agriculture is estimated in kilograms or tons per hectare per year. Natural biomass production varies greatly from region to region and climate to climate. It can reach up to 20 tons/hectare (t/ha) in tropical regions. To produce bioethanol, on one hectare per year, the following quantities of raw material can be obtained[3]:
- Wheat: 7.5 tons, or 4.2 tons of starch, then 26 hectoliters (hl) of ethanol
- Corn: 10 tons, or 6.3 tons of starch, then 40 hl of ethanol
- Beetroot: 96 t or 16 t of sugar, then 96 hl of ethanol
- Sugar cane: 90 to 110 t, or 80 to 100 t of sugar, then 90 hl of ethanol
But since water occupies a large, if not very large, share of the plant mass, both in fields and meadows and in forests, and even more so in algae, it is necessary to specify whether we are talking about natural biomass containing its water, or dry matter. This distinction concerns mainly wood, which, when freshly cut, can contain more than 50% water. But to burn well it must contain less than 20%.
The energy content of fuels is measured by their calorific value, called higher[HCV] when it includes the energy required to vaporize water, which is essential during combustion, and lower[HCV] when this latent heat is deducted.
On this basis, one ton of dry matter has an average energy content of 0.45 toe. A crop production of 10t/ha/year therefore corresponds to 4.5 toe; this is already a relatively high production. One ton of wood with 25% relative humidity contains 0.33 toe, which is still equivalent to 3,833 kWh. By way of comparison, a ton of fossil fuel, petrol, heating oil or natural gas, contains from 1 to 1.12 toe, i.e. at least 3 times more energy (Read: Energy units).
3.3. Energy efficiency
The notion of energy efficiency is a general concept, used when transforming one form of energy into another form in a machine, a living body, a fuel or fuel production process. When the manufacture of a fuel, bio or not, requires the consumption of external energy (allo-thermal process), its efficiency is generally lower than that obtained in the opposite case.
But it is also necessary to take into account the nature, renewable or not, of this external energy.
Energy professionals call the Energy Index (Ie) the ratio between the energy released as a final product (fuel) and the fossil energy consumed.
When plants are available to produce energy, we know how much energy per hectare they contain. This is how much gross energy production (Pb) is available. It is calculated in toe/ha/year.
But the cultivation of plants (tillage, fertilizer production), their harvesting (use of tractors, machines) and especially the transformations allowing to pass from the primary resource to the final product (solid fuels, heat, biogas or fuels) require a lot of energy consumption, today fossil, according to an EF quantity. The energy value of the final product is reduced accordingly. This is also evaluated in toe/ha/year, the net production Pn = Pb – EF (toe/ha/year) can then be calculated.
This manufacturing process, after harvesting, therefore introduces yield losses, depending in particular on the processes used. This is the case, for example, for the biological production of biogas by methanization, or the thermochemical production of synthesis gas. An energy yield RE = Pn / Pb of this plant, expressed in %, can then be defined.
From an economic point of view, the calculation of energy efficiency, and therefore the calculation of costs, could also take into account the recovery, or not, of co-products resulting from the production of the final product, co-products such as cake, grains and beet pulp.
More generally, this method of calculation based on life cycle analysis (LCA) gives quite variable results, mainly because of the consideration of the renewable or non-renewable quality of the gray energy used. As a result, it can produce disputed results: 42% in France, 10% in the United States for ethanol derived from wheat, corn or beet, i.e. after the starch or sugar molecule has been transformed into biofuel. However, it is considered that, on average, the energy recovery in the form of ethanol from these cereals leads to an energy yield of 40%, with the use of non-renewable energies therefore absorbing 60% of the primary resource
4. Valorization of biomass
The transformations carried out to enhance the value of biomass bring economic value to the resources processed, but require consumption of materials (water, inputs) and energy, thus absorbing other utilitarian resources, which have a cost and environmental impact. They are also almost always carried out in several stages during which the material is either constructed or deconstructed.
4.1. Construction and deconstruction of matter: “Cycle in M”
In concrete terms, this process can be symbolically and temporally illustrated by an “M-Cycle” in which four main phases take place successively, within a “Time/entropy” diagram representative of the “order and disorder” of the material (Figure 6):
- a growth phase of the plant, natural (wild) or cultivated (agricultural) during which the plant is built and differentiated: its order grows, therefore its entropy decreases
- a deconstruction phase (sawing of wood, crushing of bulbs, grinding of grains, preparation of pulp or hydrolysis, for example) during which its order decreases and its entropy increases; it then provides material such as wood, flour or glucose
- a construction phase of the final product such as furniture, bread, paper or biofuel
- a consumption phase (destruction), in which the material of the product is generally decomposed (e. g. food or fuel combustion) and thus deconstructed again.
The first stage of the “M” being that of the creation of the resource (growth of the plant), and the last being that of its consumption, stages 2 and 3 of the recovery correspond successively to a first and a second transformation. To manufacture wood pellets, tree trunks are first deconstructed by sawing, crushing and drying, and then the pellets are manufactured by high-pressure molding, without glue or binder. Similarly, bioethanol is produced by grinding and extracting glucose from beets or sugar cane, followed by fermentation and distillation. All these operations generate residues or waste, which sooner or later return to nature or are recovered and reprocessed; the M above, looped back, is therefore topologically a cycle.
Waste recovery itself represents a vast economic sector with many diverse sectors. It is part of the circular economy, enshrined in the law on energy transition, but also in a new principle of biomass development: that of cascading transformations (Figure 7). In this important concept, residues are not considered as waste but as new work materials called co-products, recoverable, whose transformations will create residues themselves likely to become recoverable.
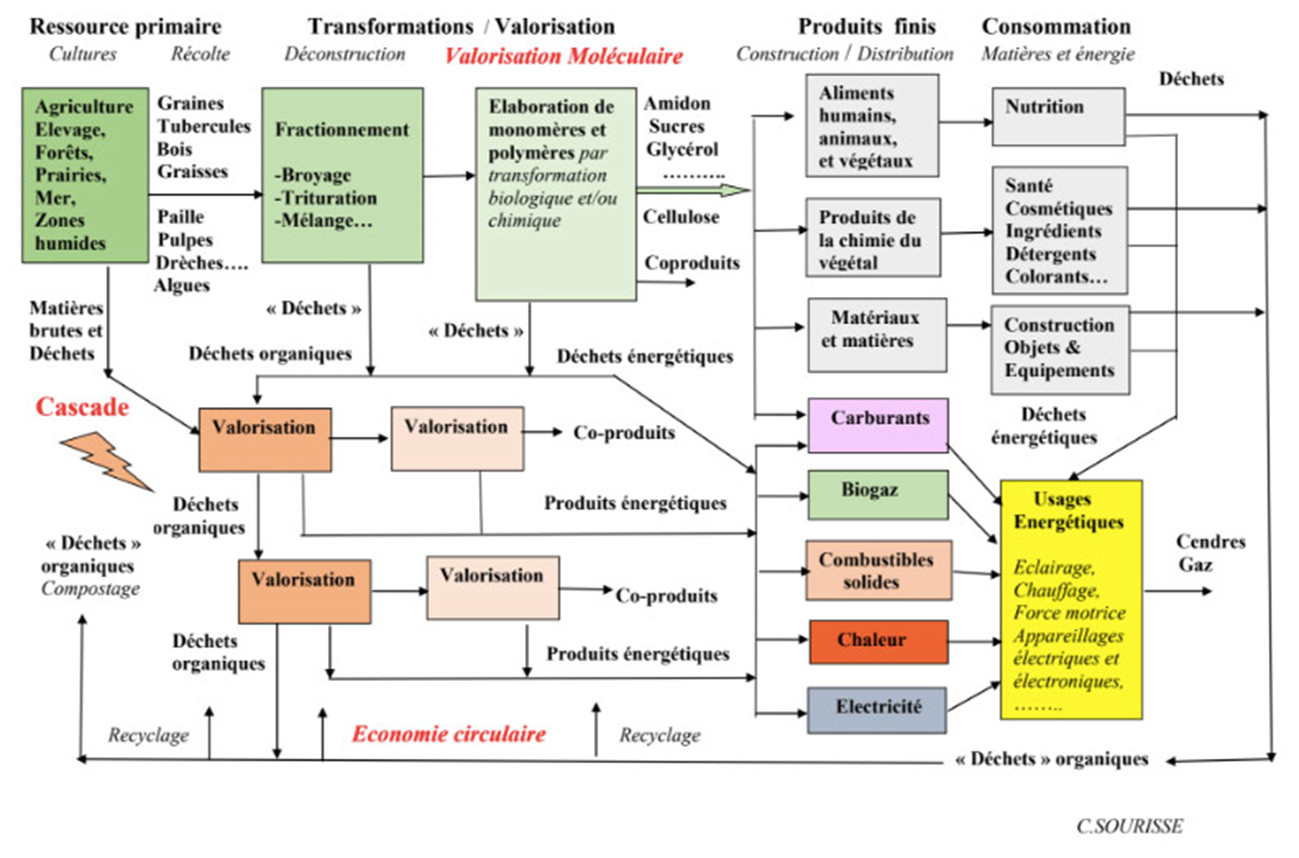
Fig. 7. Structure of a plant chemistry chain (platform molecule), with its cascading outlets for waste and energy products. [Source: Association de chimie du vegetal & C.SOURISSE]
In addition, at the end of the cascade, real waste, technically considered as such, retains an economic value because it generally still contains energy (olive stones burned in factory boilers, for example). The circular economy is thus called upon to play a particularly important role in the management of energy in a region or country.
4.2. Value chains and biosourced production system
From an economic point of view, construction-deconstruction transformations take place within sectors in which various actors create or maintain the primary resource (farming, forestry), produce agro-resources (industries), cultivate or harvest seafood products (fishing, aquaculture), then elaborate the final products (industrial products of all kinds). In past centuries, these so-called traditional sectors were by nature “monoflux” because they were organized around a main flow of material linking farmer, forest engineer or fisherman to the final consumer. This was the case for the production of logs, which simply consisted of cutting the trunks from the trees, sawing them and delivering them to private users for combustion.
But biology and chemistry have gone through this, and a triple vision of plants has taken precedence over their only external perception: that of the massive resource (plant in its natural state), of cells and fibers (histological vision) and that of their molecules. New properties with significant economic opportunities have emerged, making it possible to multiply the final products from the same resource. They have made it possible to develop new tree sectors based on plant chemistry and biotechnology (Figure 8).
The same plant, maize for example, can be used to feed poultry, make polenta, produce starch for pharmacies or paper mills, biogas, industrial alcohol or bioethanol incorporated into car gasoline!
In this case, the tree structure of the transformations is top-down, and is based on one or more primary resource(s) but on a single “platform molecule” with molecular diversification towards multiple markets.
On the other hand, a fuel manufacturer can also produce biodiesel from a multitude of oilseed crops: sunflower, rapeseed, palm, all kinds of animal fats, or algae. In the latter case, the tree structure of the supply chain(s) leading to this fuel is upward: a single market, but multiple primary resources.
5. Final energy products and their uses
Ultimately, in the production of biosourced energy, there are a plurality of sectors based on agricultural, forestry, marine and waste treatment resources, resulting in various forms of final energy sources: solid biofuels (wood logs, pellets, charcoal), biogas, biofuels and electricity.
5.1. Solid biofuels: wood logs and pellets
Wood is mainly used for heating purposes, domestic or collective, hence the designation of wood energy. Many of its users burn logs directly from the trunk cuts made in the forests. They come from a variety of species, hardwood or resinous, who’s PCI depends on the density of the variety and especially on the moisture content of the wood used. If it is dry, its calorific value is almost identical for all species (between 4 and 4.5 kWh/Kg).
The complete combustion of wood, initially with a higher calorific value (HCV), requires the vaporization of the water it contains. This absorbs some latent heat, which reduces the amount of useful heat, with the residual calorific value becoming lower calorific value (LCV). A wood considered dry with a moisture content of 20% can lose half its useful heat if it is green.
The fuel combustion process takes place in several stages. The energy released by a heater filled with a load of wood varies with time. Thus a device with a nominal power of 20 kW, for example, can reach a maximum power of 50 kW over a relatively short period of time, and then gradually decrease over a few hours.
The thermal efficiency of combustion depends on the fuel consumed but above all on the heating appliance used, which is why more and more efficient devices and appliances, inserts or stoves are available for domestic heating.
In addition to the use of fuel in the form of logs, heating needs are increasingly being met by materials from the first or second transformation of trees. They are fuels of industrial origin, made up either of residues, bark or chips, or of chips and pellets manufactured for combustion in individual or collective thermal equipment such as wood-fired boilers.
Pellets are solid biofuels in the form of cylinders, obtained by compacting sawdust or wood chips (Figure 9). Their energy density is high, especially if they are made from softwood. They are sold in bags, or in bulk. Today, they are manufactured by a large number of suppliers. Their main characteristics are as follows: PCI of 4.7 to 5.3 kWh/kg (2 kg of pellets is therefore equivalent to 1 liter of fuel oil); energy density four times higher than that of forest chips; water and dust content below 10%; ash content below 5%.
Finally, there are solid wood biofuels, which are waste wood, such as packaging residues, pallets and other clean carriers. They come from workshops and factories in the wood sector, distribution establishments, shops or transport companies. Unusable for purposes other than the supply of energy, they are suitable for use in boilers and similar equipment.
The domestic use of firewood in fireplaces, boilers and stoves accounts for a very large share of wood energy consumption in France (Table 2). About 6 million households, or nearly one in four homes, use this heat source. However, a relatively rapid renewal and modernization of the equipment fleet leads these users to use pellets, which improves the energy efficiency of their installation. A range of combined “wood and solar thermal” solutions could be developed jointly for individuals or inhabitants of new collective housing of limited size (Table 2).
Tableau 2:Biomass energy in France’s energy balance in 2015.
Primary consumption (EC) | Mtep | % of EC | Final consumption (CF)
|
Mtep | % of CF |
Wood | 9,8 | 3,9 | Individual heating | 6,5 | 4,3 |
Biofuels feedstock | 3,1 | 1,2 | Collective heating | 2,5 | 1,7 |
Household waste | 1,0 | 0,4 | Biofuel | 2,5 | 1,7 |
Agr. residues | 0,3 | 0,1 | Biogas | 0,1 | 0,05 |
Electricity | 0,5 | 0,3 | |||
Total | 14,2 | 5,6 | 12,1 | 9,05 | |
Source: Ministry of Energy Env. Key figures 2016. Electricity production includes 0.45 Mtoe of biogas and 0.5 Mtoe of wood and waste, some of which is cogeneration. The % are calculated on a primary consumption of 253 Mtoe and a final consumption of 150 Mtoe. |
The use of boilers in collective boiler rooms, often operating with platelets, combined with heating networks (mainly district heating) is a much more efficient solution in terms of efficiency. This solution is particularly well suited to heating public, industrial or agricultural premises (dehydration of fodder, heating of greenhouses or stables).
5.2. Solid fuels: charcoal
Charcoal is a fuel that has been used on a very large scale throughout the world for many centuries. It has the advantage of offering a high calorific value (~8 kWh/kg) compared to that of wood (~5 kWh/kg), in a form that is easier to transport and handle than logs and other branches of firewood, which is particularly heavy when it is still wet.
Its traditional manufacture is also easy to make, in wheels set in the middle of nature. Their operation is based on the execution of a pyrolysis producing a carbon-rich fuel, leaving ashes.
The wood is piled up there and then covered with a cover to prevent it from burning completely in the open air. However, the latter is allowed to partially penetrate below; the manufacturing operation in the enclosure successively involves drying the wood (20° to 110°), pre-carbonization (110° to 270°), then exothermic decomposition releasing various gases from the vapors and tar (270° to 400°), all completed by high temperature refining (400° to 500°).
Burned in a high-efficiency fireplace (25% for a conventional stove, in particular), charcoal provides a useful energy of 2 kWh/kg, ~3 times more than that from 5 times more wood (Figure 10).
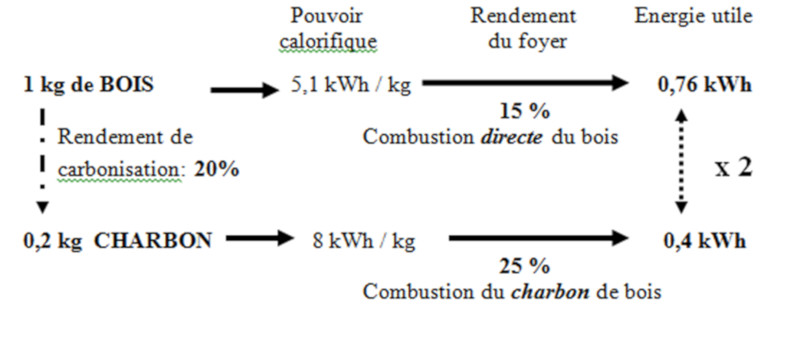
Fig 10. Yields on the production and use of firewood and charcoal. [Source: Gromard Christian and Louvel Roland, La biomasse en Afrique, AFD]
From the same quantity of plant material, the energy yield of charcoal is therefore about twice as low as that of wood, which means that twice as many trees must be felled to obtain the same amount of heat. If the forests exploited for this purpose are not regenerated, artisanal charcoal production is therefore economically negative and territorially destructive. However, in various developing countries, particularly in Africa, Latin America and South-East Asia, the use of charcoal, manufactured and used though this technique remains widespread, with a world consumption of about 50 million tons!
However, charcoal production could be carried out in an industrial environment on a large scale, with good yield and under suitable ecological conditions in regards to forestry that would conserve primary resources. Roasting techniques also exist, improving the heating capacity of the fuels obtained.
Finally, it is worth mentioning, among the solid energy products with a promising future, fuel briquettes obtained by agglomeration of plant residues, mainly agricultural residues. They can indeed offer a high energy content but they require the use of a binder, and have a lower calorific value than charcoal.
5.3. Biogas by methanization or methanation
The production of biosourced energy can also be based on the transformation of all kinds of waste, plant, animal and human (household waste) into biogas, rich in methane, and therefore in calories, called renewable gas or sometimes “green gas”. In 2017, it represents only a very small share of gas consumption in France (0.1%), which could however increase to 30% in 2030.
This gas can be obtained through two channels:
- by biological means, via the degradation of agricultural residues, animal or human excreta from micro-organisms, in fermentation units: this is then a methanization process.
- by thermochemical means, using pyro-wood/carbon gasification techniques, applying instead to lignocellulosic materials in wood or its residues; these transformations lead to the manufacture of chemical compounds such as synthesis gases, mixtures of carbon monoxide and hydrogen, with multiple outlets; the process used, based on methanization, is then called ” wood/carbon gasification”.
5.3.1. The methanization of organic, vegetable and animal matter
The methanization of plants and organic matter is a natural biological fermentation process that takes place in a closed environment, known as anaerobic fermentation. It occurs spontaneously when wet organic waste is contained, for example in a bag or container. Biogas, partially composed of methane, is thus created, accompanied by a rise in temperature.
This gas being combustible can provide heat, which can be transformed into electricity or to obtain a renewable gas that can be injected into a grid or a gaseous biofuel (biomethane).
This fermentation of the biomass is carried out in a “digester-methanizer”, which produces a mixture of methane and carbon dioxide, rich in energy, as well as a mixed liquid and solid residue: the digestate. Its liquid phase can be used as nitrogen fertilizer, its solid phase as agricultural amendment to compost (Figure 11).
Various types of methanization installations exist, including large industrial methanization plants:
- some large cattle farms treat their livestock manure at the farm site itself, with clear support from the agricultural authorities
- waste storage centers (landfills), legally required to capture their biogas to avoid their release without combustion into the atmosphere, use this gas by combustion in boilers or even by transforming it into biofuel
- effluent or urban waste treatment sites (sewage sludge, most often) or industrial sites (agri-food production units, pulp mills) also use this biomass by methanization (Read: Methanization: from wastewater treatment to biogas injection into the network).
Territorial methanization centers, which treat various residues (mowing, pruning, slurry or manure waste) in a central digester, are also operating. However, these installations, which are often expensive and often raise profitability problems, are only implemented on large capacity sites. They also do not eliminate the need to store about 50% of biomass tonnages in landfills.
Methanization plants generally use 50% livestock manure, 25% carbonaceous agricultural substrates and 25% exogenous bio-waste. Farm manure is a real yeast of methanization, but it is not very methanogenic and must therefore be supplemented by carbonaceous substrates.
An agricultural project of this type costs on average ~1 million euros (M€) for a power supply of ~150 kW (from 50 to 1000 kW), with a total energy efficiency of about 65%. Heat is generally not used much, except for the process itself and the energy of the buildings, but the digestates produced are recovered, because the liquid phase is a nitrogen fertilizer, the solid phase can be used as compost.
5.3.2. Biogas by methanation or wood/carbon gasification
Gasification is a relatively complex operation, carried out on mineral or organic carbonaceous materials (coal, hydrocarbons) or biomass, in order to produce a biogas called synthesis gas, which is combustible but is mainly used for chemical transformations, in particular to produce hydrogen (Read: Green hydrogen production). Syngas is a highly recoverable product, composed mainly of carbon monoxide (CO) and hydrogen (H2).
In this operation, known as thermochemical, the biomass is transformed into a gas with energy by means of a preliminary drying, then a preparation phase, which then takes place in several stages, at high temperature. It gives rise to a pyrolysis reaction followed by combustion producing the heat necessary to dry the work material and allow the gasification of the products. It takes place in a reducing environment: just enough oxygen is added to provide the necessary energy to activate the wood/carbon gasification reactions. Biofuels can also be produced with this gas (Figure 12).
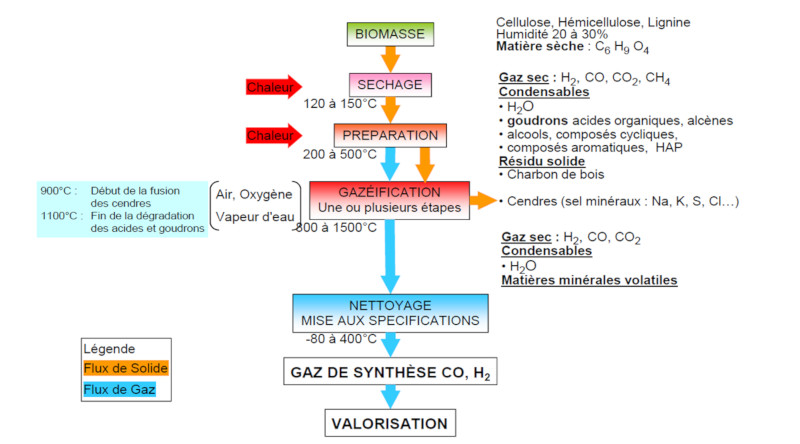
Fig. 12. Synthesis gas manufacturing process by gasification. [Source: Guillaume Boissonnet, CEA, SEM Master Course, INPG 2012]
This syngas can be injected into a distributor’s grid or used on-site to produce heat or heat and electricity in co-generation.
5.4. Bio-fuels
Biofuels are not new. Since ancient times, and probably before, people had discovered the calorific value of vegetable oils, since they used oil lamps and knew the energetic virtues of alcohol, now called ethanol. When Rudolf Diesel invented the combustion engine at the beginning of the last century, he used an oil derivative, but the ethanol engine was also invented.
It was only towards the end of the 20th century that large farmers, who had become agro-industrial, but also oil companies, understood that cereals, sugar beet and oilseed products (rapeseed, sunflower) could be major sources of energy and therefore enable biofuel production (Figure 13). But the automotive sectors could not provide new 100% green engines, technologically different from those running on fossil fuels; moreover, there was no question of massively diverting agricultural production from food markets. This is why the biofuel-hydrocarbon mixture solution, with a low proportion of biofuel (10%), has been adopted in Europe, unlike Brazil, which has opted for engines running on 85% ethanol, extracted from abundant and cheap sugar cane.
As in the food sector, where there are chains based on polysaccharides (starch and sugar), oils and fats (oilseeds, protein crops), two main chains dominate biofuel production: ethyl alcohol (ethanol), and its derivative ethyl tertiary butyl ether (ETBE), and methyl vegetable oil ether (VOME) or biodiesel. The first supplies petrol engines, the second diesel engines. The use of biofuels is highly beneficial from an ecological, economic and strategic point of view, as it significantly reduces CO2 emissions to the atmosphere, opens up opportunities for surplus agricultural production and contributes to countries’ energy independence.
These sectors, which are now mature and developed on a large industrial scale, are however called “first generation” because they are based on so-called traditional agricultural resources, and are therefore ultimately subject to competition for use: they not only threaten the supply of food to rapidly growing populations, but also compete with resources of oil origin, at fluctuating prices.
This is why the development of 2nd generation chains has been undertaken: it is a question of extracting the energy contained in the non-food part of plants, and therefore of their ligno-cellulosic components. In practice, this consists in transforming cellulose molecules into glucose, which is then subjected to fermentation to produce ethanol. Technically possible, the development of this approach is hampered by the problem of very low initial yields, and therefore requires the development of efficient enzymatic and metabolic processes based on new enzymes and yeasts.
In favor of such developments, biotechnological processes have a major advantage over traditional chemistry, in particular thermo-chemistry; the treatments are carried out on heterogeneous and unpurified substrates, under operating conditions close to natural, i.e. in diluted aqueous suspensions, at low temperature, and under atmospheric pressure. These advantages are nevertheless limited by a number of disadvantages related to the complexity of the processes, the stabilization of the strains and the difficulty of managing the fermentation operations.
5.4.1. Bioethanol
Depending on the nature of the biomass, bioethanol can be produced by various processes (Figure 14). Cereal seeds (1st generation) can either be ground and liquefied, before undergoing saccharification, followed by fermentation, distillation and dehydration. Another process consists in producing starch milk in liquid phase by centrifugation, then hydrolyzing it to extract glucose, which is then treated by fermentation, distillation and dehydration.
If the source biomass consists of sugar cane or beet, the sugar must first be extracted, separating it from its substrates such as bagasse or beet pulp, and then, as before, treated by fermentation, distillation, and dehydration (Table 3).
When the biomass used is ligno-cellulosic (2nd generation), bioethanol is manufactured according to the same principles: after mechanical pre-treatment (crushing), hydrolysis is carried out under the action of enzymes to produce a fermentable substrate, then subjected to yeasts. After this fermentation, the resulting product is distilled to extract the ethanol.
This process therefore requires the production of enzymes (from bacteria and fungi), and the availability of appropriate yeasts. These enzymes and yeasts are difficult to select and exploit if we want to benefit from high yield and large-scale production conditions, using, in addition, various raw materials; this is why this process, developed in particular at Pomacle Bazancourt (Futurol project), is still in the pre-industrial stage.
Tableau 3: Yields and costs of bioethanol production from various plants.
Plant resource | Gross quantity harvested
(tons) |
Platform product | Processed final product: ethanol (hl) | Production cost excluding co-product valuation
(€/liter) |
Production cost with valorization of co-products
(€/liter) |
Beetroot | ~96 | ~16 tons of sugar | ~ 96 | 0,60 | 0,50 |
Sugar cane | ~ 90 to 110 | ~12 to 16 tons of sugar | 80 to 100 | 0,20
|
|
Corn | 10 | 6,3 tons of starch | ~ 40 | 0,75 | 0,50 |
Wheat | 7,5 | 4,2 tons of starch | ~ 26 | 0,75 | 0,50 |
Source: France Agrimer. |
To perfectly adapt bioethanol to the operating constraints of engines, oil companies react it chemically with a well-known product, isobutene, to obtain the ETBE molecule. This is the product found in 10% of the E10 fuel distributed by petrol pumps. However, it should be noted that at equal mass, the calorific value of bioethanol is only 0.64 times that of lead-free super.
5.4.2. Biodiesel
Biodiesel can be made from oilseed crops, either on a large scale or on the basis of cultivated plants, which differ from region to region: sunflower and rapeseed in Europe, maize mainly used in the United States, soybean and palm kernel oil in Asia (product derived from the fruit stones of oil palm trees, their pulp providing palm oil).
In all cases, the oils from these plants must first be extracted by crushing, followed by filtration. The waste from this operation is oilcake, which is energetic and rich in protein, and is highly valued particularly for animal feed. The crude oils are then semi-refined by a degumming and neutralization treatment.
To make them usable in engines, the oils are then chemically treated by one or the other of two distinct processes:
- trans-esterification, with methanol, which provides an oil methyl ester (OME) and is useful for the production of lubricants and solvents; this reaction also provides glycerol, a platform product widely used in plant chemistry.
- a hydrogenation that removes unwanted oxygen atoms.
It is also interesting to note that 1st generation biodiesel can also be made from animal fats, and even waste oils. There is also a 2nd generation biodiesel sector, using dedicated plants (grasses) or wood waste. In this case, a thermochemical process is used. It involves pre-treatment by roasting, followed by gasification and purification, followed by a Fischer Tropsch reaction. The calorific value of biodiesel is close to that of traditional “diesel”, with 1 ton of EMHV ester equivalent to 0.9 tons of diesel.
5.4.3. Algae and cyanobacteria
They are another potential source of biofuels.
Algae and cyanobacteria are photosynthetic organisms with particularly interesting properties: they fix about half of the carbon dioxide absorbed in the entire biosphere, while their biomass production per unit area is several times higher than that of terrestrial plants. They could therefore play an essential role in the resource mix of the future.
The living environment of plankton (phytoplankton and zooplankton), of which there are thousands of species, forms the basis of the food chain of all marine organisms: fish and other seafood products, consumed in large quantities. But there are also many algae and aquatic animals in fresh waters (rivers or lakes) and aquaculture pools.
Food outlets for fisheries and aquaculture products already play a major role in the global economy, but what is of even greater interest to researchers and industrialists today are the materials found in these algae. Already used for a long time for biochemical (pharmaceutical) and cosmetic uses, some of them contain molecules that are particularly rich in poly-saccharides, proteins, polyphenols, lipids and mineral salts.
In energy use, microalgae are extremely interesting, because their surface productivity is potentially very high:50,000 liters of oil per hectare, compared to 6,000 liters for palm oil! In the future, using natural or artificial photosynthesis processes, these organisms could convert CO2 from the atmosphere or from the CO2 collected at the exit of large plants, in dedicated installations, off-shore and off-land for agricultural purposes, in brackish or alkaline basins or lagoons operating directly or indirectly with solar energy. Energy-rich carbon molecules, particularly biofuels, could be developed (Figure 15).
But we have yet to reach this stage. Although pre-industrial test and demonstration stations have been actively developing and testing high-efficiency cultivation and extraction processes for energy materials (mainly oil) for several years, these prospects are still distant (at least 20 years) on a regional or national economy scale.
5.5. Generation and co-generation of bio-sourced electricity
The energy recovery of biomass can go as far as electricity generation whenever an advantageous fuel cost (60€ / per ton delivered) added to the avoided CO2 price (15€ / per ton delivered) makes thermoelectricity-biomass very competitive. This is the case for industries and communities that increasingly use wood or straw-fired boilers (3,000 existing sites growth: 5 to 10% / year), some of which are associated with co-generation facilities.
Cogeneration is the simultaneous production of two different energies in the same process. The most frequent case is the production of electricity and heat, with heat coming from electricity production or the reverse. In practice, the wood is burned in the furnace of a boiler, which produces high-pressure steam that drives the axis of a turbine. This axis in turn drives an alternator, which produces electricity. The low-pressure water vapor from the turbine passes through a condenser, which provides heat (Figure 16).
A good example of this type of installation is provided by the pulp mill in Saint Félicien, Quebec. The supply, in cogeneration-biomass, of 1 MW of electrical power implies, on average, the prior production of 5 MW of thermal energy, i.e. the use of 6 MW PCI in primary-biomass energy capacity taking into account a boiler efficiency of 85%. For an operation of 7,000 H per year, or 42,000 MWh PCI supplied, the installation therefore requires, for 1MWe of power supplied, the annual combustion of approximately 14,000t of fresh cellulosic biomass.
1. Wood preparation
2. Boiler 3. Smoke treatment 4. Turbine 5. Alternator 6. Condenser 7. Heat network |
6. Bioenergy in the energy transition
Particularly since the last decades of the 20th century, energy transition has become a “burning obligation” (Read: Energy transition, a major challenge for the planet). To avoid further global environmental degradation, including serious climate risks, all countries are called upon to make their economic development as sustainable as possible. In terms of energy supply, this objective means greater control of demand, through greater moderation where possible and more efficient use of energy, and a reorientation of supply towards energy sources that are less polluting and emit less greenhouse gases (GHG) than fossil fuels. What role should bioenergy play in the energy balances that could ensure an energy transition?
6.1. Advantages of bioenergy
Given the massive use of fuelwood in countries that are still less industrialized, this position is already by far the most important in the world. It is expected to grow further and win new countries in view of the many advantages of this energy resource:
- the plant mass, on a global scale, although very unevenly distributed, is extremely abundant; the annual production of cellulose, the main component of wood, is about 100 billion tons, therefore at least twenty times higher than that of oil; to this resource is added the mass of organic waste (agricultural and industrial residues, garbage, green waste) that can be economically and ecologically recovered; in France, they represent about 600 million tons/year.
- in contrast to the use of fossil fuels, which will soon be exhausted, biomass is indefinitely renewable, because on our human scale, solar energy will always be present, as will water and carbon dioxide, provided that their quality and the major natural balances are respected (in thirty years, world vegetation has increased by 14%!).
- bioenergy from this biomass is very diversified: solid fuels in the form of pellets, gaseous or liquid, can easily be distributed in multiple forms: bulk, bags, heating networks, gas tanks or pump distribution; in addition, all can be converted into electricity, with or without co-generation.
- ecologically (carbon cycle), biomass is totally virtuous: it absorbs as much CO2 through photosynthesis as it releases through the combustion of living beings and combustion in all its forms; its carbon footprint is therefore neutral.
- the production of bioenergy is also ecologically advantageous because its transformation processes take place at low temperatures, such as methanization on the farm or the production of bioethanol.
- the combustion of low-carbon biogases is also much less polluting in fine particles than that of liquid hydrocarbons.
- always from an ecological point of view, this biomass is by nature biodegradable; it therefore leaves no organic waste in the short to medium term, mineral components (metals) can also be recovered for agronomic purposes.
- economically, in the context of the circular economy, its valuation can be integral; according to the cascade principle, the whole plant (fruits, stems, leaves, trunks, bark) can be processed, thus providing added value, and this at the place of production, without transport and with the creation of local jobs.
- last but not least, unlike most renewable sources, biomass resources are storable and can even store, after conversion, those from intermittent sources such as power-to-gas.
6.2. Disadvantages to be considered
Faced with all these advantages, which advocate in favor of a very wide use of bioenergy, several limitations may take form in some countries more than in others, from the following disadvantages:
- the difficulty of access to resources, particularly in areas with low plant density but also in mountain forests, as well as problems of transporting heavy materials such as logs or even low density materials such as coppices or herbaceous plants.
- additional operating costs due to the economic difficulty of extracting energy from wet plants and organic materials (animal droppings, sludge) soaked in water, as well as those of processing and consuming gray energy throughout the biogas and biofuel production chain, mainly in the case of low-energy plants or agricultural and forestry waste.
- the fact that all combustion processes emit GHGs, even if allowing plants to grow back over a period of a few years, their carbon cycle is neutral.
- the risks of market shortages or imbalances, particularly in food markets, due to competition for use, as in the case of German or American farmers growing maize solely to produce biogas or ethanol.
- with regard to biofuels, ethanol or biodiesel, not all engines are yet able to use them at 100%.
Ultimately, a precise assessment of the advantages of each bioenergy in relation to its potential competitors will be the only way to make the decision. It is however likely to be favorable in many situations, particularly in countries rich in biomass, but still very poor in the use of modern and efficient energy sources (Read: Energy supply to off-grid African populations).
Bibliography
Les ressources du futur issues du monde végétal – C.Sourisse – 408 p – March 2017 – Editor: Covabis www.covabis.fr
Biocarburants / Cinq questions qui dérangent – J.P.Legalland – J.L.Lemarchand – 2018 – Editions Technip
Les triples A de la bio-économie: Efficacité, sobriété, et diversité de la croissance verte – 294 p – 2012 Editor: L’Harmattan (Edited by Claude Roy – Le Club des Bio-économistes).
La biomasse, énergie d’avenir – Hervé Bichat et Paul Mathis – 225 p. – Mars 2013 – Editor : Editions Quae
Bioraffinerie 2030 – Une question d’avenir (PomacleBazancourt) –Collective work. – 252 p. – Sept 2014 – Editor: L’Harmattan
Energies renouvelables en agriculture – Bernard Pellecuer – 2015 – Editor : Editions France Agricole
‘’Rapport de l’OPECST/ Assemblée nationale -Sénat- De la biomasse à la bioéconomie : une stratégie pour la France’’ – 194 p – 10 February 2016 -(Public hearing on 25 June 2015)
‘’Une stratégie bioéconomie pour la France : Plan d’action 2018-2020’’ (12-page brochure) Ministère de l’agriculture – Février 2018
References
[1] Fossil or non-biodegradable materials are not legally biomass.
[2] Source: H. Bichat and P. Mathis
[3] Source: France Agrimer